The journey into the history of nuclear fusion starts in the early 20th century. Scientists were curious about how stars, including our Sun, produced such vast amounts of energy. The British physicist Arthur Eddington was one of the first to suggest that stars might be powered by nuclear fusion, combining hydrogen atoms to form helium and releasing energy in the process.
This idea was revolutionary because it proposed a new form of energy production that was vastly different from chemical reactions like burning coal or wood. The concept of fusion was further explored over the years, and it expanded beyond just an inquiry into stellar power. It began to encompass a broader investigation into the nature of matter and energy.
The first human-made nuclear fusion occurred not for peaceful purposes but in warfare. In 1952, during the Ivy Mike hydrogen bomb test, scientists achieved self-sustaining nuclear fusion. This was a thermonuclear explosion, not a controlled reaction suitable for energy production.
The quest for controlled nuclear fusion began earnestly after this, with various experiments and reactor designs being tested. In 1958, a significant milestone was reached with the theta-pinch device Scylla-I, which achieved a true controlled thermonuclear fusion reaction². Around the same time, different types of fusion reactors were developed, including the stellarator and tokamak, which used magnetic fields to contain hot plasma and achieve fusion conditions.
What Is Nuclear Fusion?
Nuclear fusion is a process where two light atomic nuclei combine to form a heavier nucleus, releasing energy in the process.
Nuclear fusion is a nuclear reaction where two light atomic nuclei merge to form a single heavier nucleus. This process is accompanied by the release of energy. It’s the same kind of reaction that powers the sun and other stars, where hydrogen atoms fuse to form helium.
In more scientific terms, during fusion, the nuclei of two atoms come so close that the nuclear forces overcome their electrostatic repulsion (the force that normally keeps them apart because they are both positively charged) and bind together. This usually requires conditions of extremely high temperature and pressure.
The energy released during fusion comes from a small amount of mass from the nuclei that is converted into energy, as described by Einstein’s famous equation ( E=mc^2), where (E) is energy, (m) is mass, and (c) is the speed of light. This means that even a tiny amount of mass can be converted into a huge amount of energy.
Fusion reactions are the source of immense power and are what make stars shine so brightly. On Earth, scientists are trying to replicate these reactions to create a new source of clean energy.
How Does Nuclear Fusion Take Place?
Nuclear fusion takes place under extreme conditions of temperature and pressure, which are necessary to overcome the natural repulsion between the positively charged nuclei of atoms. Here’s a step-by-step explanation of the process:
- Heating Up: Atoms must be heated to incredibly high temperatures, typically millions of degrees Celsius. This gives the atomic nuclei enough kinetic energy to move at high speeds.
- State of Plasma: At these high temperatures, electrons are stripped from their atoms, creating a state of matter known as plasma, which is a hot, charged gas consisting of free electrons and nuclei.
- Overcoming Repulsion: The positively charged nuclei repel each other due to the electrostatic force. However, if they collide with enough energy (from the heat), they can come close enough for the strong nuclear force to take over. This force, which works only at very short distances, is much stronger than the electrostatic force and can bind the nuclei together.
- Fusion Reaction: When two nuclei collide and stick together, they form a heavier nucleus. This process releases a tremendous amount of energy because some of the mass of the original nuclei is converted into energy (following Einstein’s equation (E=mc2).
- Energy Release: The energy released in fusion comes out as kinetic energy of the particles produced in the reaction, including any new nuclei and any released neutrons. This energy can then be harnessed for various uses if controlled effectively.
Electrons During Fusion:
During nuclear fusion, the intense heat causes atoms to collide with such force that their nuclei can overcome the electrostatic repulsion and fuse together. This high-energy environment also strips electrons away from their atoms, creating a plasma—a hot, ionized state of matter composed of free-moving nuclei and electrons.
In this plasma state, electrons are not bound to any particular nucleus, unlike in a typical atom. They move freely within the plasma, contributing to its overall charge and helping to balance the charge of the positively charged nuclei.
While the nuclei are undergoing fusion and combining to form heavier elements, the free electrons continue to move about in the plasma. They play a crucial role in conducting electricity and heat within the plasma and can affect the stability and confinement of the plasma in a fusion reactor.
In short, during fusion, electrons become part of the plasma and are not directly involved in the fusion reaction itself, which only involves the atomic nuclei. So, nuclear fusion occurs when two light atomic nuclei combine to form a heavier nucleus under extreme conditions, releasing a significant amount of energy in the process.
Nuclear Fusion in the Sun
The Sun, like other stars, is a natural fusion reactor where hydrogen nuclei fuse to form helium under extreme temperatures and pressures at its core. The core of the Sun is incredibly hot and dense. Temperatures reach about 15 million degrees Celsius, and the pressure is immense due to the Sun’s gravity.
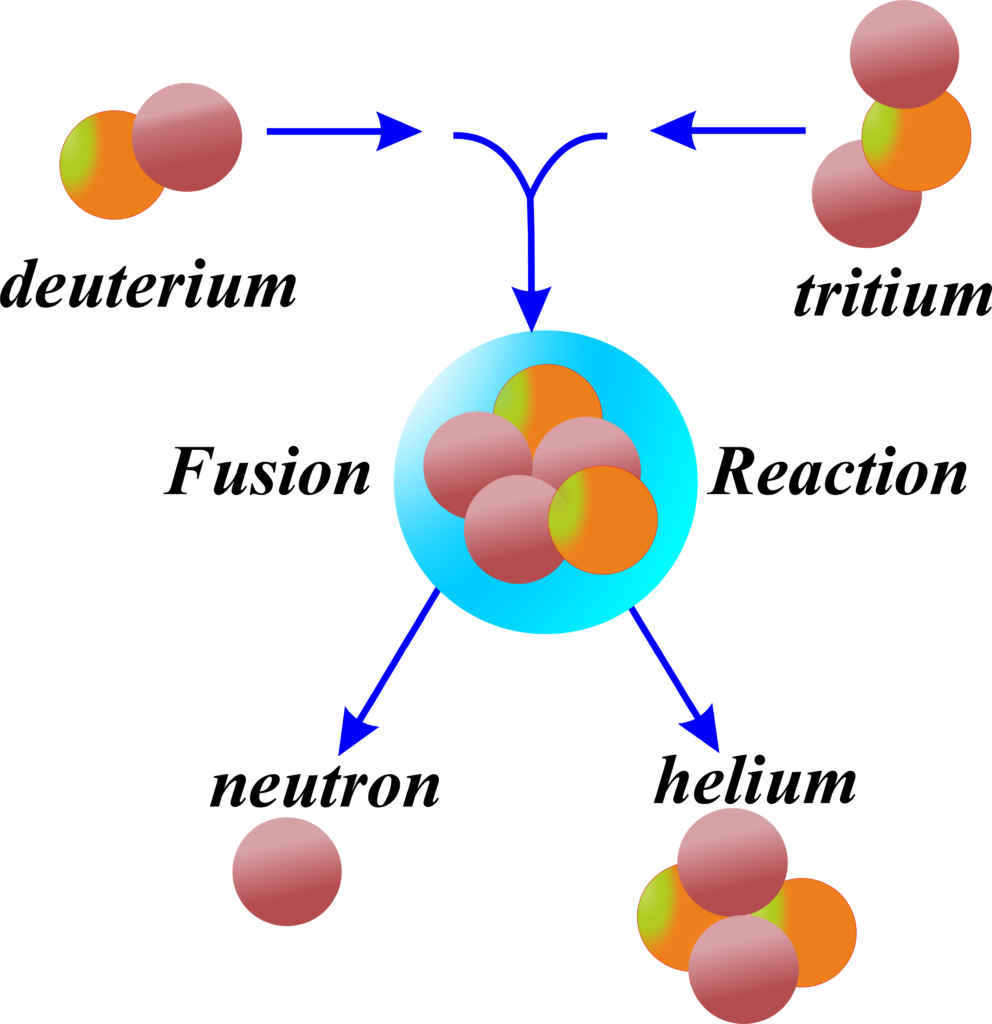
The Sun’s primary fusion process involves hydrogen atoms. Under extreme conditions in the core, hydrogen nuclei (protons) collide with such force that they can fuse. The most common fusion reaction in the Sun is the proton-proton chain. This process involves several steps:
Two protons fuse to create a deuterium nucleus (one proton and one neutron), releasing a positron and a neutrino. The deuterium nucleus then fuses with another proton to form helium-3 (two protons and one neutron), releasing a gamma-ray photon. Two helium-3 nuclei collide and fuse to form helium-4 (two protons and two neutrons), releasing two protons back into the plasma.
Each step in these reactions releases energy in the form of gamma rays, neutrinos, and kinetic energy of the particles. The gamma rays eventually make their way to the surface of the Sun and are emitted as sunlight.
This fusion process is continuous and produces the energy that powers the Sun and provides light and warmth to our solar system. Nuclear fusion in the Sun converts hydrogen into helium, releasing energy that sustains the Sun’s glow and supports life on Earth.
Fusion Reaction in the Sun
The sun generates energy through a series of nuclear fusion reactions known as the proton-proton (pp) chain. This process converts hydrogen into helium under the extreme temperatures and pressures at the sun’s core.
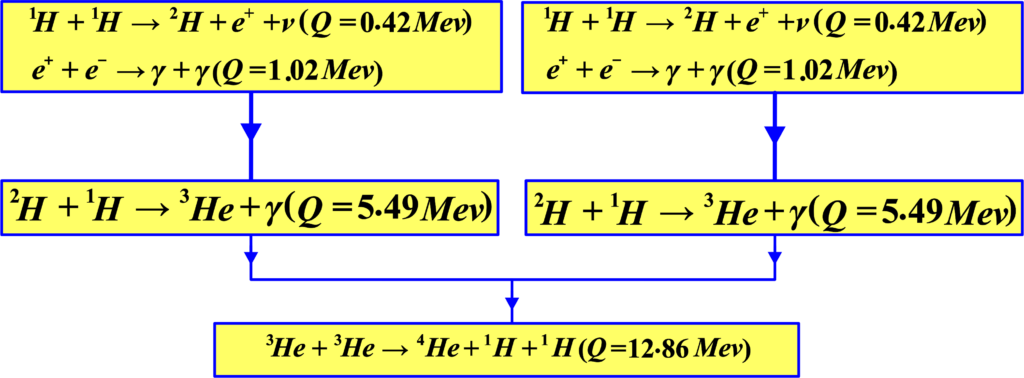
Proton-Proton Chain: The most common fusion reaction in the sun starts with two hydrogen nuclei (protons) fusing to form a deuterium nucleus, a positron (the antimatter counterpart of an electron), and a neutrino:
\(\displaystyle \text{p} + \text{p} \rightarrow {}^2_1\text{H} + \text{e}^+ + \nu \)
Deuterium Fusion: The deuterium nucleus then quickly fuses with another proton to form helium-3 and releases a gamma-ray photon:
\(\displaystyle {}^2_1\text{H} + \text{p} \rightarrow {}^3_2\text{He} + \gamma \)
Helium Formation: Two helium-3 nuclei collide and fuse to form helium-4, releasing two protons in the process:
\(\displaystyle {}^3_2\text{He} + {}^3_2\text{He} \rightarrow {}^4_2\text{He} + 2\text{p} \)
The energy released in these reactions is carried away by the gamma rays, positrons, neutrinos, and kinetic energy of the particles. The gamma rays eventually reach the sun’s surface and escape as sunlight.
The overall reaction converts four protons into one helium-4 nucleus, with mass converted into energy according to Einstein’s equation ( E=mc2 ). This energy is what we see as sunlight.
Neutrinos Produced During Solar Fusion:
Neutrinos are fascinating particles produced in vast quantities during nuclear fusion in the Sun. Here’s what happens to them:
Neutrinos are produced as a byproduct of the nuclear reactions that power the Sun, particularly during the proton-proton chain reaction. Neutrinos interact very weakly with matter, which means they can pass through the Sun and other celestial bodies without being absorbed or deflected.
Because of their weak interaction, neutrinos produced in the Sun’s core escape almost immediately and travel through space at nearly the speed of light. Some of these solar neutrinos reach Earth, where they can be detected by specialized instruments like underground neutrino observatories. These detectors often use large volumes of water or other substances to increase the chance of a neutrino interacting and being observed.
Applications of Nuclear Fusion
Nuclear fusion, the process that powers the stars, has several potential applications here on Earth:
- Energy Production: The most prominent application of nuclear fusion is in generating electricity. Fusion reactors aim to replicate the Sun’s energy production, providing a nearly limitless source of power without the long-lived radioactive waste associated with current nuclear fission reactors.
- Medical Isotopes: Fusion can be used to produce medical isotopes, which are used in diagnostic imaging and cancer treatment. These isotopes are currently produced in nuclear reactors or particle accelerators, but fusion could offer a new method.
- Research: Fusion research contributes to our understanding of plasma physics, materials science, and energy production, which can have broader applications in various scientific and technological fields.
- Space Exploration: Fusion could potentially be used for propulsion in space travel, offering a more efficient and faster way to send spacecraft on long-distance missions.
While these applications are promising, it’s important to note that controlled nuclear fusion for practical use is still under development and not yet realized on a commercial scale. Scientists and engineers around the world are working to overcome the technical challenges to harness this clean and abundant source of energy.
Disadvantages of Nuclear Fusion
Challenges include achieving and maintaining the extreme conditions needed for fusion, and handling and disposing of materials exposed to intense neutron radiation. While nuclear fusion has many potential benefits, there are also several disadvantages to consider:
- Technical Challenges: Achieving controlled nuclear fusion on Earth is extremely difficult. It requires creating and maintaining conditions similar to the Sun’s core, which involves very high temperatures and pressures that are challenging to produce and contain.
- High Costs: The technology needed for fusion reactors is complex and expensive. Building and operating a fusion reactor requires significant investment, and it’s still uncertain whether fusion can be made cost-effective for electricity production.
- Material Durability: The intense environment inside a fusion reactor can cause significant wear and tear on materials, leading to challenges in finding materials that can withstand heat and radiation over long periods.
- Radioactive Waste: Although much less than fission reactors, fusion reactors still produce some radioactive waste from the materials that become irradiated during the fusion process.
- Research and Development Time: Developing practical fusion power has taken decades and may take several more before it becomes a reality. This long development time is a disadvantage when considering the urgent need for clean energy solutions.
- Neutron Radiation: Fusion reactions produce high-energy neutrons that can be difficult to shield against and can activate materials, making them radioactive.
Nuclear Fusion Reactor
A nuclear fusion reactor is a device designed to harness the power of nuclear fusion, the process that powers the sun and stars, for energy production on Earth. Here’s what you need to know about it:
The goal of a fusion reactor is to create the conditions necessary for fusion to occur—extremely high temperatures and pressures—so that nuclei can overcome their natural repulsion and fuse together, releasing energy.
In a fusion reactor, fuel (usually isotopes of hydrogen like deuterium and tritium) is heated to form a plasma, which is a hot, ionized gas where electrons are separated from nuclei.
The plasma must be confined long enough for fusion to occur. This is challenging because the plasma is extremely hot and can’t be contained by any physical material. Two main methods are used for confinement:
- Magnetic Confinement: Uses powerful magnetic fields to hold the plasma in place, as in tokamaks or stellarators.
- Inertial Confinement: Uses lasers or ion beams to compress small pellets of fuel to achieve the necessary conditions for fusion.
If fusion occurs successfully, the energy released can be used to heat water, produce steam, and drive turbines to generate electricity. Fusion reactors are currently in the experimental stage. Scientists and engineers are working on making them practical and efficient enough for widespread use as a clean energy source.
Types of Nuclear Fusion Reactor
Magnetic Confinement:
Magnetic confinement fusion reactors use powerful magnetic fields to contain and control the hot plasma needed for nuclear fusion. Here are the main types:
- Tokamak: The most well-known and researched type of magnetic confinement reactor. It has a donut-shaped design (toroidal) and uses a combination of toroidal and poloidal magnetic fields to confine the plasma. The ITER project in France is an example of a tokamak.
- Stellarator: Similar to a tokamak, but with a more complex design that twists the plasma into a helical shape. This design aims to create a more stable plasma without the need for a large current through the plasma, which can be problematic in tokamaks. The Wendelstein 7-X in Germany is an example of a stellarator.
- Reversed Field Pinch (RFP): A variation where the magnetic field is reversed in the center of the device, which helps to stabilize the plasma against certain instabilities.
- Spherical Tokamak: A compact version of the tokamak with a spherical shape rather than a donut shape, which may allow for smaller, less expensive reactors.
- Field-Reversed Configuration (FRC): A design where the magnetic field lines are reversed so that they point towards the center of the reactor, creating a stable configuration for the plasma.
How do magnetic fields help confine the plasma in a tokamak?
In a tokamak, magnetic fields are crucial for confining the plasma, which is necessary for nuclear fusion to occur. Here’s how they work:
Plasma consists of charged particles—ions and electrons—that are influenced by magnetic fields. By applying a magnetic field, these charged particles can be directed and contained.
A tokamak has large magnets that wrap around the donut-shaped chamber to create a toroidal (ring-shaped) magnetic field. This field causes the charged particles in the plasma to spiral around the field lines, keeping them from touching the walls of the reactor.
Additional magnets, called poloidal field coils, are placed inside and outside the toroidal chamber to create a second magnetic field that wraps around in the short direction of the donut. This field helps to stabilize the plasma and keep it in the center of the chamber.
The combination of toroidal and poloidal magnetic fields creates a “magnetic cage” that confines the plasma effectively. The charged particles follow the twisted magnetic field lines, moving in helical paths.
A current is induced in the plasma itself, which generates its own magnetic field and contributes to the overall confinement.
The precise control of these magnetic fields is essential for maintaining a stable, confined plasma where fusion can occur. It’s a delicate balance because any instability can cause the plasma to touch the reactor walls, cooling it down and stopping the fusion reaction.
Each type has its own advantages and challenges, and research continues to determine which design—or combination of designs—might be best suited for practical fusion energy production.
Inertial Confinement
Inertial confinement fusion (ICF) reactors aim to achieve nuclear fusion by rapidly compressing and heating small fuel pellets. Here are the main types:
- Laser-Driven ICF: This method uses powerful lasers to heat and compress a small pellet containing fusion fuel. The National Ignition Facility (NIF) in the US is an example where numerous laser beams are focused onto the pellet, causing its outer layer to explode and drive the remaining material inward, increasing pressure and temperature to initiate fusion.
- Ion Beam-Driven ICF: Instead of lasers, ion beams are used to compress and heat the fuel pellet. This method is less developed than laser-driven ICF but offers some potential advantages, such as more uniform compression.
- Z-Pinch: This technique uses strong electric currents in a plasma to generate magnetic fields that compress a cylindrical fuel target. The Z machine at Sandia National Laboratories is an example of this approach.
- Magnetic Target Fusion (MTF): A hybrid approach that combines aspects of magnetic confinement with inertial confinement. A small magnetized target is compressed by an external driver, such as a laser or ion beam, to achieve fusion conditions.
How do lasers compress the fuel pellet in inertial confinement fusion?
Each type of ICF reactor uses different methods to compress and heat the fuel, but they all share the goal of creating the extreme conditions necessary for nuclear fusion in a very short time frame. In inertial confinement fusion (ICF), lasers are used to compress the fuel pellet through a process called “implosion.” Here’s how it works:
The fuel pellet is typically a small sphere containing fusion fuel, usually isotopes of hydrogen like deuterium and tritium. A series of high-energy laser beams are precisely targeted to hit the outer surface of the fuel pellet from all directions at the same time.
When the laser beams strike the pellet’s surface, they rapidly heat it, causing the outer layer to ablate, or vaporize explosively. This ablation creates a reaction force that drives the remaining part of the pellet inward.
As the outer layer explodes outward, it pushes the rest of the material inward, compressing it to a fraction of its original size. This compression raises the temperature and pressure inside the pellet to extreme levels.
If the compression is sufficient, conditions within the core of the pellet can reach those required for nuclear fusion—temperatures of millions of degrees and high pressure—allowing the nuclei to overcome their electrostatic repulsion and fuse together, releasing energy.
The entire process happens incredibly quickly, in a matter of nanoseconds, and must be perfectly synchronized to achieve uniform compression and efficient fusion.
Q.What are some challenges in achieving uniform compression for ICF reactors?
Achieving uniform compression in inertial confinement fusion (ICF) reactors is challenging due to several factors:
Laser Precision: The lasers must hit the fuel pellet with extreme precision and uniformity. Any slight variation in timing, energy, or angle can lead to asymmetrical compression, reducing the efficiency of the fusion reaction.
Pellet Quality: The fuel pellet must be perfectly spherical and free of any imperfections. Flaws in the pellet can cause irregularities in compression.
Instabilities: During compression, various hydrodynamic instabilities can occur, such as Rayleigh-Taylor and Richtmyer-Meshkov instabilities, which can mix the fuel and disrupt the implosion symmetry.
Plasma Effects: The plasma created by the ablation process can interfere with the incoming laser beams, affecting their ability to deliver energy uniformly to the pellet.
Material Properties: Understanding and controlling the behavior of materials under the extreme conditions of ICF is difficult. Materials may behave differently at high pressures and temperatures, affecting compression.
Diagnostic Challenges: It’s challenging to diagnose what happens during the implosion because it occurs over such a short timescale and under extreme conditions, making it hard to adjust and improve the process.
Researchers are working on advanced technologies and techniques to address these challenges and achieve the uniform compression necessary for efficient fusion reactions in ICF reactors.
Nuclear Fusion Reactor Working
A nuclear fusion reactor works by replicating the process that powers the sun and stars, where atomic nuclei combine to form heavier nuclei, releasing energy. Here’s a simplified explanation of how it works:
Fuel Preparation: The reactor uses a mixture of hydrogen isotopes, typically deuterium and tritium, as fuel. These isotopes are more easily fused than regular hydrogen because they have extra neutrons.
Heating: The fuel is heated to extremely high temperatures, often several times hotter than the sun’s core, to create a plasma—a state of matter where electrons are stripped from their atoms, leaving behind positively charged ions.
Confinement: The plasma must be confined long enough for fusion to occur. This is done using magnetic fields in magnetic confinement reactors (like tokamaks) or by compressing the fuel pellet rapidly in inertial confinement reactors (like those using lasers).
Conditions for Fusion: For nuclei to fuse, they must overcome their natural repulsion (since they are both positively charged). High temperature gives them enough energy to collide with sufficient force, while high pressure brings them close enough together.
Fusion Reaction: When conditions are right, the nuclei can fuse to form a new, heavier nucleus. For example, deuterium and tritium can fuse to form helium and a neutron. This process releases a tremendous amount of energy.
Energy Extraction: The energy released from fusion reactions is primarily in the form of kinetic energy of the neutrons. This energy can be absorbed by the reactor walls and used to heat water, produce steam, and ultimately generate electricity through turbines and generators.
The working of a nuclear fusion reactor involves creating and maintaining the right conditions for fusion to occur and then harnessing the energy released from these reactions to produce power.
Also Read: Nuclear Fission
Applications of Nuclear Fusion Reactor
Nuclear fusion reactors have the potential to provide a clean, safe, and virtually limitless source of energy. Here are some of the key applications:
- Electricity Generation: The primary application of nuclear fusion reactors is to generate electricity. Fusion reactors could provide a more sustainable and environmentally friendly alternative to fossil fuels and current nuclear fission reactors.
- Reduced Radioactive Waste: Fusion reactors produce less long-lived radioactive waste than fission reactors, making them a cleaner option for power generation.
- Abundant Fuel Supply: The fuel for fusion, isotopes like deuterium and tritium, is abundant. Deuterium can be extracted from seawater, and tritium can be bred from lithium, which is also relatively abundant.
- No Greenhouse Gas Emissions: Fusion reactions do not produce greenhouse gases, a major contributor to climate change.
- Energy Security: Fusion reactors could reduce dependence on imported fuels and contribute to energy security for countries currently relying on foreign energy sources.
- Space Exploration: Compact fusion reactors could potentially be used to power spacecraft for long-duration space missions, providing a reliable energy source far from Earth.
- Medical Isotope Production: Fusion reactors could be used to produce medical isotopes for diagnostic imaging and cancer treatment.
- Research and Development: Fusion technology advances our understanding of plasma physics and high-energy physics, contributing to scientific knowledge and innovation.
While nuclear fusion reactors are not yet commercially viable, their potential applications are vast and could have significant impacts on energy production and various industries in the future.
Solved Examples
Problem 1: Calculate the energy released in the fusion reaction where two deuterium nuclei fuse to form a helium-3 nucleus and a neutron. Given the atomic masses are:
- Deuterium (\(\displaystyle ^2_1 \text{H} \)) = 2.014102 u
- Helium-3 (\(\displaystyle ^3_2 \text{He} \)) = 3.016029 u
- Neutron (n) = 1.008665 u
Solution: The fusion reaction can be represented as:
\(\displaystyle ^2_1 \text{H} + ^2_1 \text{H} \rightarrow ^3_2 \text{He} + n \)
The total mass before fusion:
\(\displaystyle m_{\text{before}} = 2 \times 2.014102 \, \text{u} = 4.028204 \, \text{u} \)
The total mass after fusion:
\(\displaystyle m_{\text{after}} = 3.016029 \, \text{u} + 1.008665 \, \text{u} = 4.024694 \, \text{u} \)
The mass defect (∆m ):
\(\displaystyle \Delta m = m_{\text{before}} – m_{\text{after}} = 4.028204 \, \text{u} – 4.024694 \, \text{u} = 0.00351 \, \text{u} \)
Convert mass defect to energy (1 u = 931.5 MeV):
\(\displaystyle E = \Delta m \times 931.5 \, \text{MeV/u} = 0.00351 \times 931.5 \, \text{MeV} = 3.27 \, \text{MeV} \)
The energy released in the fusion of two deuterium nuclei to form a helium-3 nucleus and a neutron is approximately 3.27 MeV.
Problem 2: Calculate the total energy produced in the Sun by the fusion of 1 kg of hydrogen into helium. Given the mass of hydrogen (proton) is 1.007825 u, the mass of helium-4 is 4.002603 u, and the Sun fuses four protons to form one helium-4 nucleus.
Solution: The fusion reaction in the Sun is:
\(\displaystyle 4 \, ^1_1 \text{H} \rightarrow ^4_2 \text{He} + 2e^+ + 2\nu_e \)
Total mass before fusion:
\(\displaystyle 4 \times 1.007825 \, \text{u} = 4.0313 \, \text{u} \)
Total mass after fusion:
\(\displaystyle 4.002603 \, \text{u} \)
Mass defect (∆ m):
\(\displaystyle \Delta m = 4.0313 \, \text{u} – 4.002603 \, \text{u} = 0.028697 \, \text{u} \)
Energy released per helium-4 nucleus (1 u = 931.5 MeV):
\(\displaystyle E = 0.028697 \times 931.5 \, \text{MeV} = 26.73 \, \text{MeV} \)
Number of helium-4 nuclei produced from 1 kg of hydrogen:
\(\displaystyle \text{Number of protons} = \frac{1 \, \text{kg}}{1.007825 \, \text{u}} \times 6.022 \times 10^{26} \, \text{atoms} \)
\(\displaystyle = 5.978 \times 10^{26} \, \text{protons} \)
The number of helium-4 nuclei produced:
\(\displaystyle = \frac{5.978 \times 10^{26}}{4} = 1.4945 \times 10^{26} \)
Total energy produced:
\(\displaystyle E_{\text{total}} = 1.4945 \times 10^{26} \times 26.73 \, \text{MeV} \)
\(\displaystyle = 3.995 \times 10^{27} \, \text{MeV} \)
Convert MeV to joules (1 MeV = 1.60218 × 10-13 J):
\(\displaystyle E_{\text{total}} = 3.995 \times 10^{27} \times 1.60218 \times 10^{-13} \, \text{J} \)
\(\displaystyle = 6.402 \times 10^{14} \, \text{J} \)
The total energy produced in the Sun by the fusion of 1 kg of hydrogen into helium is approximately (\(\displaystyle 6.402 \times 10^{14} \, \text{J} \)).
Problem 3: Explain the significance of the Lawson Criterion in the context of nuclear fusion reactors and calculate the minimum product of plasma density and confinement time required for deuterium-tritium fusion to be viable.
Solution: The Lawson Criterion states that for a fusion reactor to produce net energy, the product of the plasma density (n) and the energy confinement time (τ) must exceed a certain value. For deuterium-tritium (D-T) fusion, the criterion is typically:
\(\displaystyle n \tau \geq 10^{20} \, \text{s/m}^3 \)
For D-T fusion to be viable:
\(\displaystyle n \tau \geq 10^{20} \, \text{s/m}^3 \)
If we assume a plasma density (n) of (\(\displaystyle 10^{20} \, \text{particles/m}^3 \)):
\(\displaystyle \tau = \frac{10^{20}}{10^{20}} = 1 \, \text{s} \)
The Lawson Criterion requires that the product of the plasma density and the confinement time for deuterium-tritium fusion be at least (\(\displaystyle 10^{20} \, \text{s/m}^3 \)). For example, with a plasma density of (\(\displaystyle 10^{20} \, \text{particles/m}^3 \)), the confinement time must be at least 1 second.
Problem 4:Calculate the Q-value (energy released) for the fusion reaction where two tritium nuclei fuse to form a helium-4 nucleus and two neutrons. Given the atomic masses are:
- Tritium (\(\displaystyle ^3_1 \text{H} \)) = 3.016049 u
- Helium-4 (\(\displaystyle ^4_2 \text{He} \)) = 4.002603 u
- Neutron (n) = 1.008665 u
Solution: The fusion reaction can be represented as:
\(\displaystyle 2 \, ^3_1 \text{H} \rightarrow ^4_2 \text{He} + 2n \)
The total mass before fusion:
\(\displaystyle m_{\text{before}} = 2 \times 3.016049 \, \text{u} = 6.032098 \, \text{u} \)
The total mass after fusion:
\(\displaystyle m_{\text{after}} = 4.002603 \, \text{u} + 2 \times 1.008665 \, \text{u} = 6.019933 \, \text{u} \)
The mass defect (∆m):
\(\displaystyle \Delta m = m_{\text{before}} – m_{\text{after}} = 6.032098 \, \text{u} – 6.019933 \, \text{u} = 0.012165 \, \text{u} \)
Convert mass defect to energy (1 u = 931.5 MeV):
\(\displaystyle E = \Delta m \times 931.5 \, \text{MeV/u} = 0.012165 \times 931.5 \, \text{MeV} = 11.33 \, \text{MeV} \)
The Q-value (energy released) for the fusion reaction of two tritium nuclei to form a helium-4 nucleus and two neutrons is approximately 11.33 MeV.
Problem 5: Describe the basic working principle of a Tokamak fusion reactor and calculate the magnetic field required to confine a plasma with a density of (\(\displaystyle 10^{20} \, \text{particles/m}^3 \)) and temperature of (\(\displaystyle 10^8 \, \text{K} \)). Assume the major radius of the Tokamak is 1.5 meters.
Solution: Working Principle;
A Tokamak is a type of nuclear fusion reactor that uses magnetic fields to confine plasma in a doughnut-shaped (toroidal) chamber. The magnetic fields are produced by external coils and an induced plasma current, which together create a helical magnetic field that keeps the plasma stable and away from the reactor walls.
Calculation:
\(\displaystyle \beta = \frac{2\mu_0 n k_B T}{B^2} \)
– ( \(\displaystyle\beta \)) is the plasma pressure ratio (assumed to be 0.1 for high-confinement mode),
Rearranging for (B):
\(\displaystyle B^2 = \frac{2\mu_0 n k_B T}{\beta} \)
\(\displaystyle B = \sqrt{\frac{2 \times 4\pi \times 10^{-7} \times 10^{20} \times 1.38 \times 10^{-23} \times 10^8}{0.1}} \)
\(\displaystyle B = \sqrt{\frac{2 \times 4\pi \times 10^{-7} \times 1.38 \times 10^5}{0.1}} \)
\(\displaystyle B = \sqrt{\frac{1.738 \times 10^{-1}}{0.1}} \)
\(\displaystyle B = \sqrt{1.738} \)
\(\displaystyle B \approx 1.32 \, \text{T} \)
The magnetic field required to confine a plasma with a density of (\(\displaystyle 10^{20} \, \text{particles/m}^3 \)) and temperature of (\(\displaystyle 10^8 \, \text{K} \)) in a Tokamak with a major radius of 1.5 meters is approximately 1.32 Tesla.
Problem 6: Determine the energy gain factor (Q) for a fusion reactor if the input power required to maintain the plasma is 20 MW and the output power from the fusion reaction is 500 MW.
Solution: The energy gain factor (Q) is defined as the ratio of the power output from the fusion reaction to the power input required to maintain the plasma:
\(\displaystyle Q = \frac{P_{\text{output}}}{P_{\text{input}}} \)
Given:
– (\(\displaystyle P_{\text{output}} = 500 \, \text{MW} \))
– (\(\displaystyle P_{\text{input}} = 20 \, \text{MW} \))
\(\displaystyle Q = \frac{500 \, \text{MW}}{20 \, \text{MW}} = 25 \)
The energy gain factor (Q) for the fusion reactor is 25.
FAQs
What is nuclear fusion and how does it differ from nuclear fission?
Nuclear fusion is the process where two light atomic nuclei combine to form a heavier nucleus, releasing a significant amount of energy. This contrasts with nuclear fission, where a heavy nucleus splits into lighter nuclei. Fusion typically occurs under extremely high temperatures and pressures, as found in the cores of stars, whereas fission can occur at relatively lower temperatures and is commonly used in nuclear reactors and atomic bombs.
How does nuclear fusion take place?
Nuclear fusion takes place when two light nuclei, such as isotopes of hydrogen (deuterium and tritium), come close enough for the strong nuclear force to overcome their electrostatic repulsion. This typically requires extremely high temperatures (millions of degrees) and pressures to give the nuclei sufficient kinetic energy to collide and fuse. When they fuse, they form a heavier nucleus and release a large amount of energy.
How does nuclear fusion occur in the Sun?
In the Sun, nuclear fusion occurs in the core where temperatures and pressures are extremely high. The primary fusion process is the proton-proton chain reaction, where hydrogen nuclei (protons) fuse to form helium. This process releases vast amounts of energy in the form of light and heat, which powers the Sun and provides energy to our solar system.
What is a nuclear fusion reactor and what is its purpose?
A nuclear fusion reactor is a device designed to replicate the conditions necessary for nuclear fusion to occur, with the aim of harnessing the energy produced. The purpose of a fusion reactor is to create a controlled environment where light nuclei can fuse, releasing energy that can be converted into electricity. Fusion reactors aim to provide a nearly limitless, clean, and safe source of energy.
What are the different types of nuclear fusion reactors?
There are several types of nuclear fusion reactors, with the most notable being:
- Tokamak: A toroidal (doughnut-shaped) device that uses magnetic fields to confine the hot plasma necessary for fusion.
- Stellarator: Similar to a tokamak but with a more complex magnetic field configuration to confine the plasma.
- Inertial confinement fusion (ICF): Uses lasers or other means to compress and heat small fuel pellets to achieve fusion.
- Magnetized target fusion (MTF): Combines aspects of magnetic confinement and inertial confinement.
How does a nuclear fusion reactor work?
A nuclear fusion reactor works by creating the extreme conditions needed for fusion, typically involving high temperatures and strong magnetic or inertial confinement. In a tokamak, for example, hydrogen isotopes are heated to form a plasma, which is then confined using powerful magnetic fields. The plasma must be maintained at the right temperature, pressure, and confinement time for fusion to occur. The energy released from fusion reactions is then captured and converted into electricity.
What are the challenges in developing nuclear fusion reactors?
The challenges in developing nuclear fusion reactors include achieving and maintaining the extremely high temperatures and pressures needed for fusion, effectively confining the plasma, handling the materials that can withstand such extreme conditions, and making the process energy-efficient and economically viable. Additionally, managing the byproducts and ensuring the overall safety of the fusion reactor are critical challenges that researchers are working to overcome.