In the 18th century, the invention of the steam engine was a pivotal moment. It was the workhorse of the Industrial Revolution, transforming industries and transportation. But, it was not very efficient. Scientists and engineers wanted to make it better, and their efforts led to the birth of thermodynamics as a formal science.
In the 1820s, a French military engineer named Sadi Carnot pondered over the efficiency of steam engines. He published a work called “Reflections on the Motive Power of Fire,” describing the idealized engine that could do the most work with a given amount of heat energy. This concept laid the groundwork for the first and second laws of thermodynamics.
Throughout the 19th century, other scientists like James Joule, Lord Kelvin, and Rudolf Clausius contributed significantly. Joule demonstrated converting mechanical work into heat, leading to the conservation of energy principle. Kelvin and Clausius further refined the laws, introducing concepts like absolute zero temperature and entropy.
The principles of thermodynamics have since been applied to various technologies, from internal combustion engines to refrigerators. It’s a field that has not only explained the behavior of steam engines but also laid the foundation for modern physics, including quantum mechanics and relativity.
What is a Thermodynamic System?
A thermodynamic system is a defined space or matter within the universe that we are observing, which allows us to apply the principles of thermodynamics to understand how energy and work interact within this space. This could be anything from a single atom to a complex machine. It’s where we observe and measure changes, such as energy transfer or work done.
Imagine you’re in a kitchen. You have a pot of water on the stove, and you’re about to boil it to make some tea. Now, in the world of physics, especially in the chapter on thermodynamics, we like to simplify things to study them better. So, we draw an imaginary line around the pot of water. This line marks the boundary of our interest, and everything inside it is our thermodynamic system.
A thermodynamic system is a specific portion of the universe that we’ve chosen to study. It’s like selecting a character in a video game; this is the character (system) you’re going to play with and understand. In our kitchen example, the pot of water is the system because we’re interested in how it behaves when we heat it.
Now, why do we even bother with this? By focusing on a particular system, we can observe and measure things like temperature changes, energy entering or leaving the system, and even the work done by the system (like when the water starts to boil and moves the lid). These observations help us understand the fundamental laws of physics that govern how energy is transferred and transformed.
A thermodynamic system is like a toolkit that helps us understand how things like energy, heat, and work behave in the physical world. Think of it as a specific part of the universe we want to study, like focusing on one tool in a big toolkit. This system can be something simple, like air trapped in a syringe, or more complicated, like a whole factory process.
Scientists and engineers use these systems to watch and measure how energy changes in different situations. Whether it’s a tiny system or a massive one, studying these systems is the starting point for learning about thermodynamics, which is like the science of how energy moves and changes. So, when you hear about a thermodynamic system, just picture a special part of the universe we’re investigating to understand how things like energy play out.
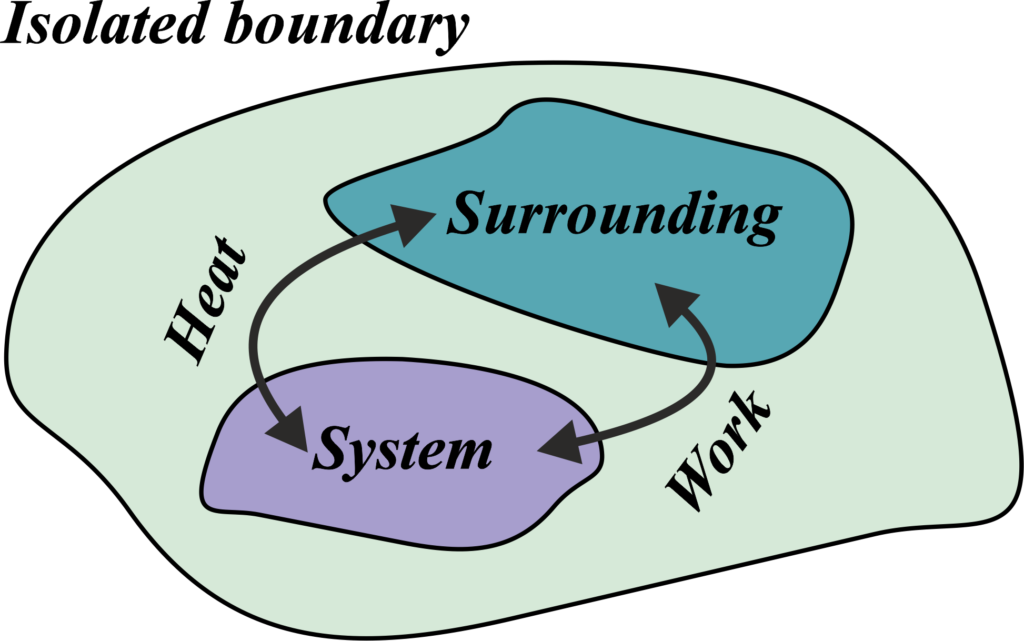
The concepts of system, surroundings, and system boundary are fundamental to understanding energy interactions in thermodynamics.
System
A system in thermodynamics is the specific part of the universe that we’re examining. It’s where we apply our scientific curiosity to uncover how energy flows and does work. It’s our window into the vast and intricate dance of particles, forces, and energy that make up everything around us.
In the realm of thermodynamics, the term “system” refers to any part of the universe that we’ve decided to study and observe. It’s like selecting a specific character in a video game; this is the character you control and learn about. In thermodynamics, the system is the “character” we’re interested in understanding.
Let’s say you’re in a park, and you decide to focus on a soccer ball. That ball becomes your system. You can watch how it moves, how it interacts with the ground, and how it reacts when someone kicks it. You’re not concerned with the entire park, just the ball. Similarly, in thermodynamics, you might choose a gas inside a cylinder or the steam in a power plant as your system.
The system is defined by the questions we want to answer or the phenomena we want to study. It can be as small as an atom or as large as a star. What’s important is that it’s the area of focus where we apply the laws of thermodynamics to see how energy and work are related within this system. Example: If you’re studying the water in a boiling kettle, the water is your system.
System Boundary
The boundary of a system is what separates it from its surroundings. It can be real, like the walls of a container, or imaginary, like the surface we imagine around a gas. Imagine you’re playing a game of soccer. The lines drawn on the ground that mark the edges of the playing field are crucial because they define where the game is played. In thermodynamics, we have something similar called a system boundary.
A system boundary is like an imaginary fence or a real physical barrier that separates the system (the thing we’re studying) from everything else, which we call the surroundings. It’s not always something you can touch or see; sometimes, it’s just a line we draw in our minds to focus on a specific part of the universe.
For example, if you’re studying a cup of hot tea, the system boundary could be the walls of the cup. This boundary tells us that we’re only interested in what’s happening inside the cup, not outside of it. It helps us to concentrate on the energy changes, like heat transfer, occurring within the tea and not worry about the rest of the room.
In physics, especially in thermodynamics, setting a clear system boundary is essential because it allows us to apply the laws of thermodynamics accurately. It helps us understand how energy moves and transforms within that defined space.
Example: A thermos flask is a great example of a system boundary in thermodynamics. It’s designed to keep your hot drinks hot and your cold drinks cold for as long as possible. But how does it do this? It’s all about the system boundary it creates.
The thermos flask acts as a closed system when it comes to matter—nothing gets in or out unless you open it. But it’s also close to an isolated system in terms of energy transfer because it’s designed to prevent heat from escaping or entering.
Here’s how it works:
- The flask has a double-walled container, and between these walls, there’s a vacuum.
- This vacuum is a space with no air, and since air is a medium through which heat is transferred, the vacuum acts as a perfect insulator.
- The walls of the flask are usually coated with a reflective material to prevent heat transfer by radiation.
The thermos flask’s boundary—the walls and the vacuum—prevents energy (like heat) from moving in or out. This makes it a nearly perfect example of an isolated system boundary in thermodynamics. It’s not perfect because, over a very long time, some heat will eventually escape, but it’s as close as we can get to everyday objects.
So, the thermos flask keeps your hot chocolate warm by not letting the heat energy escape into the cold surroundings, just like how you’d want to keep all your study energy focused and not let distractions “leak” it away.
Characteristics of Boundaries
When we talk about boundaries in thermodynamics, we’re referring to the limits that define a system. These boundaries can have various characteristics that determine how the system interacts with its surroundings. Here are some key characteristics:
1) Real or Imaginary: Some boundaries are physical, like the walls of a container, while others are just conceptual lines we draw in our minds.
- Real Boundaries: Real boundaries are physical and tangible. They’re like the walls of a classroom that separate it from the hallway. In thermodynamics, a real boundary could be the metal walls of a boiler or the glass of a thermometer. These are actual, physical things you can touch and see.
- Imaginary Boundaries: On the other hand, imaginary boundaries are conceptual. They’re like the lines of a soccer field that exist only during the game. In thermodynamics, an imaginary boundary might be the space around a cloud of steam or the surface we imagine enclosing a gas within a room. You can’t touch these boundaries, but they’re just as important for our studies.
2) Fixed or Movable: Boundaries can be stationary, like the walls of a room, or they can move, like the piston in an engine cylinder.
- Fixed Boundaries: Fixed boundaries are like the walls of your house. They don’t move. No matter what happens inside, the walls stay put. In thermodynamics, a fixed boundary means the shape and volume of the system don’t change. It’s rigid. For example, the walls of a pressure cooker provide a fixed boundary for the steam inside.
- Movable Boundaries: Movable boundaries can change position. They’re like the door of your classroom that can open and close. In thermodynamics, a movable boundary allows the system to change its volume. Think of a piston in a cylinder: when the piston moves, it changes the volume of the gas inside, which is a movable boundary.
If the boundary is fixed, the system’s volume won’t change, no matter how much pressure builds up inside. But if it’s movable, the system can do work on its surroundings by moving the boundary, like when a gas expands and pushes a piston outwards.
3) Permeable or Impermeable: This describes whether or not the boundary allows matter to pass through. A permeable boundary might be a filter or a membrane, while an impermeable boundary is sealed off, like the glass of a closed window.
- Permeable Boundaries: A permeable boundary allows substances to pass through it. Think of it like a net; water can flow through, but larger objects like fish are trapped. In thermodynamics, if a system has a permeable boundary, it means that molecules, such as gases or liquids, can move in and out of the system. This exchange could be due to processes like diffusion or osmosis.
- Impermeable Boundaries: An impermeable boundary, on the other hand, is like a solid wall. Nothing can pass through it—not water, not air, nothing. In a thermodynamic system, an impermeable boundary means that matter is contained within the system and cannot enter or leave. However, this doesn’t necessarily mean energy can’t pass through; heat might still be transferred depending on the material of the boundary.
How does an impermeable boundary affect the behavior of a gas in a container?
An impermeable boundary has a significant impact on the behavior of a gas contained within it. Since the boundary does not allow matter to pass through, the volume of the gas remains constant unless the container itself changes shape or size.
If the gas is heated, the pressure will increase because the molecules move faster and collide more frequently with the walls of the container. The impermeable boundary ensures that no gas escapes, so all the increased pressure is contained within the system.
Similarly, cooling the gas will lower the pressure inside the container as the molecular activity decreases. Again, because the boundary is impermeable, the gas cannot expand into another area to balance the pressure.
The gas cannot gain or lose mass because the impermeable boundary prevents any exchange of matter with the surroundings. This means the number of molecules in the system stays the same.
An impermeable boundary creates a closed system for the gas. This allows for the study of the gas’s behavior under various conditions without the complication of mass transfer.
4) Adiabatic or Diathermal: An adiabatic boundary does not allow heat to pass through, while a diathermal boundary does. Think of an adiabatic boundary as a perfect insulator, and a diathermal boundary as something like a metal spoon that gets hot when you leave it in a pot of soup.
Adiabatic Boundaries: An adiabatic boundary does not allow heat to pass through it. It’s like a super-insulated wall that keeps all the heat on one side. In a thermodynamic system, if you have an adiabatic boundary, it means that no heat can enter or leave the system through that boundary. This is important when we want to study how a system changes without losing or gaining heat from its surroundings.
Diathermal Boundaries: A diathermal boundary, on the other hand, allows heat to transfer across it. It’s like a window that lets sunlight warm up a room. In a thermodynamic system, a diathermal boundary means that heat can move in and out, affecting the system’s temperature and the energy within it.
These characteristics help us understand and predict how a system will behave. For example, if we know a boundary is movable and diathermal, we can expect the system to do work by moving the boundary and exchanging heat with the surroundings.
Types of Boundaries
- Adiabatic Boundary: This does not allow heat to pass through, meaning no heat exchange occurs between the system and surroundings. An adiabatic boundary does not allow heat to pass through. This means that no heat exchange occurs between the system and its surroundings. If a process happens quickly enough that no heat can be transferred, or if the system is perfectly insulated, it can be considered adiabatic. An example of an adiabatic process is the rapid compression of gas in an engine cylinder.
- Diathermal Boundary: Allows heat to pass through, permitting thermal energy exchange. A diathermal boundary, in contrast, allows heat to transfer between the system and its surroundings. This type of boundary does not restrict the flow of thermal energy, so the system can gain or lose heat. For instance, the walls of a steel container might serve as a diathermal boundary if they allow heat to pass through them.
- Rigid Boundary: Does not change its volume or shape, regardless of the changes within the system. A rigid boundary maintains a constant volume; it does not move or change shape regardless of the internal pressure or temperature changes within the system. This is typical of solid containers or structures that are strong enough to resist deformation under the conditions they are subjected to.
- Flexible Boundary: Can change its shape or volume in response to changes within the system, like pressure. A flexible boundary can move or change shape in response to changes within the system, such as pressure or temperature variations. An example of a flexible boundary is a balloon, which expands as the air inside it is heated and the molecules move more rapidly.
Surroundings
Everything outside the system boundary is the surroundings. It’s the part of the universe that can interact with the system. Imagine you’re sitting at your desk with a cup of hot tea. The tea in the cup is your system. Now, everything else around the cup—the air in the room, the desk, the rest of the house, even the outside environment—is considered the surroundings.
The surroundings are essentially the rest of the universe that interacts with your system. They can affect the system and be affected by it. For instance, the heat from your cup of tea slowly spreads into the air around it—that’s the surroundings absorbing energy from the system.
In a more technical sense, the surroundings are defined as everything outside the system that has a direct influence on the behavior of the system. It’s like the audience to a play; they don’t come onto the stage, but their reactions can influence the performance.
Example: For the boiling water, the air, the kettle’s walls, and everything outside the drawn circle are the surroundings.
How do the surroundings affect the temperature of a cup of tea?
The surroundings play a crucial role in determining the temperature of a cup of tea. Here’s how:
The primary way the surroundings affect the temperature of your tea is through heat transfer. If the room is cooler than the tea, heat will flow from the hotter tea to the cooler air. This process continues until the temperature of the tea and the surrounding air are equal.
If the cup is placed on a material that conducts heat well, like a metal table, heat will transfer out of the tea more quickly than if it were on a coaster or a wooden table. The movement of air around the cup can also affect the rate at which the tea cools. A breeze or a fan can increase heat loss through convection, cooling the tea faster.
As the tea cools, some of it will evaporate, which removes heat from the liquid and lowers its temperature. This process is more significant if the air is dry. The tea also loses heat through infrared radiation. The rate of radiative heat loss depends on the difference in temperature between the tea and its surroundings.
Also Read: Temperature
Thermodynamic System types
The characterization of thermodynamic systems comes in three main types: isolated, closed, and open systems. An isolated system, impermeable to both matter and energy exchange, maintains a constant total energy and mass. In a closed system, energy can be transferred to the surroundings while mass remains constant. Meanwhile, an open system allows for both energy and matter exchange across its boundaries. These distinctions enable the application of thermodynamic principles to a diverse array of phenomena, including the behavior of gases, the dynamics of chemical reactions, and the efficiency of engines.
Open System
An Open System in thermodynamics is a concept that allows both energy and matter to be exchanged with the surroundings. An open system allows both energy and mass to be exchanged between the system and its surroundings. Heat and work can flow across the system boundaries, and the system can perform work on its surroundings. Heat and work can flow across the system boundaries, and the system can perform work on its surroundings. The system can exchange matter with the surroundings, leading to changes in the total mass of the system. Both energy and mass are not necessarily conserved within the system; their values can change due to interactions. To understand this, let’s use a simple analogy.
Think of an open system as a garden with a fence that has gates. The plants in the garden can receive water and nutrients from outside the fence (matter), and they can also absorb sunlight and feel the wind (energy). Similarly, in an open thermodynamic system, substances like gases or liquids can move in and out, and energy in the form of heat or work can be transferred between the system and its environment.
An open system could be compared to your study group. Ideas (matter) and inspiration (energy) flow freely between group members and the outside world. This exchange helps the group (the system) to grow and develop, just like how an open thermodynamic system interacts with its surroundings.
Example: In thermodynamics, an open system allows both matter and energy to flow in and out of its boundaries.
- Boiling Pot of Water: When you boil water on a stove, the pot is an open system. The heat from the stove (energy) enters the system, and the steam (matter) escapes into the air.
- Human Body: Our bodies are also open systems. We take in food and oxygen (matter) and release carbon dioxide and waste (matter), and we exchange heat (energy) with our environment.
- Car Engine: A car engine is another example. It takes in air and fuel (matter), burns it to produce power (energy), and then expels exhaust gases (matter).
These examples show how open systems interact with their surroundings by exchanging both energy and matter, which is a key concept in understanding thermodynamic processes.
Closed System
In a closed system, energy can be exchanged with the surroundings, but there is no transfer of mass. Heat and work can flow across the system boundaries with the surroundings. The total mass of the closed system remains constant; no mass enters or exits. While mass is conserved, the total energy (internal energy, kinetic energy, potential energy) can change due to heat and work interactions.
A Closed System in thermodynamics is like a dance party in a hall with all the doors and windows closed. The music (energy) from the party can be heard outside, and the lights (also energy) can be seen from the windows, but no one (matter) can enter or leave the party once it has started.
In a more scientific sense, a closed system allows energy transfer but not matter between the system and its surroundings. It’s like a water bottle with a cap on; the water inside (matter) can’t get out, and no more water can get in, but the bottle can still get warm or cold (energy transfer) depending on the temperature outside.
A closed system helps you grasp how energy can move in and out of a system while the matter inside remains constant. This concept is key to many principles in thermodynamics, like the conservation of mass and the first law of thermodynamics, which says that energy cannot be created or destroyed, only transformed or transferred.
Example: In thermodynamics, a Closed System is one where no matter can enter or leave, but energy can be exchanged with the surroundings. Here are some examples that illustrate this concept:
- Coffee Thermos: A thermos filled with coffee doesn’t allow the liquid to escape or additional liquid to enter, making it a closed system in terms of mass transfer. However, it can still exchange heat with its surroundings, albeit very slowly due to its insulation.
- Refrigerator Refrigeration Cycle: In a refrigerator, the refrigerant circulates within a closed loop. It absorbs heat from the inside of the refrigerator (cooling the contents) and releases it to the outside environment, but the refrigerant itself doesn’t leave the system.
- Pressure Cooker: When sealed, a pressure cooker is a closed system. The steam generated from boiling water cannot escape, and no additional water can enter. The cooker can transfer heat to the surroundings when it’s hot, but the mass inside remains constant.
These examples help us understand how closed systems work by allowing energy transfer while restricting mass transfer, which is a fundamental concept in the study of thermodynamics.
Isolated System
An isolated system is a thermodynamic system that does not exchange energy or matter with its surroundings. There is no transfer of heat or work across the system boundaries with the surroundings. No exchange of matter occurs between the system and its surroundings. Both the total energy and mass within an isolated system remain constant.
An Isolated System in thermodynamics is like a space explorer in a perfectly sealed spaceship, far out in the cosmos. Just as the astronaut cannot receive supplies or send out waste, an isolated system does not exchange any matter or energy with its surroundings.
Imagine a game where you’re in a bubble that nothing can get into or out of. No matter what happens outside the bubble, it doesn’t affect you inside, and nothing you do inside the bubble affects the outside. That’s what an isolated system is like in thermodynamics. It’s completely self-contained, with no influence from or on the external environment.
Example: Water placed in a vessel that is closed as well as insulated.
In thermodynamics, an Isolated System does not exchange either energy or matter with its surroundings. Here are some examples that can help illustrate this concept:
- Thermos Flask: A well-sealed thermos flask is a classic example of an isolated system. It’s designed to prevent heat from entering or leaving, keeping your drink at the desired temperature for a long time.
- Inflated Balloon: A balloon that is tied off and does not allow air to enter or escape can be considered an isolated system. While it’s not perfect (since balloons can slowly leak air and heat), it’s a good representation of the concept.
- The Universe: The universe is often considered the ultimate isolated system because it doesn’t exchange energy or matter with anything else—it’s all there is.
Properties of Thermodynamic System
Intensive or Intrinsic Properties:
Intensive properties, also known as intrinsic properties, are characteristics of a thermodynamic system that do not depend on the system’s size or the amount of material present. These properties remain the same whether you’re looking at a drop of water or an ocean. Here are some key intensive properties:
- Temperature: It measures the average kinetic energy of particles in a system. No matter how much substance you have, the temperature is the same throughout.
- Pressure: This is the force exerted by particles as they collide with the boundaries of their container. Pressure is uniform in a system at equilibrium, regardless of its size.
- Density: It’s the mass per unit volume. Density is an intensive property because if you take two identical volumes of the same substance, they will have the same density.
These properties are fundamental in thermodynamics because they describe the state of a system independently of its mass or volume. Understanding these properties helps predict how a system will behave under different conditions without needing to know how much of the substance is present.
Extensive or Extrinsic Properties:
Extensive properties, also known as extrinsic properties, are characteristics of a thermodynamic system that depend on the size or amount of matter in the system. These properties are additive, which means if you divide the system into parts, the property’s value is the sum of its values for the parts. Here’s a closer look at some extensive properties:
- Volume (V): This is the space that the system occupies. If you have two identical systems and combine them, the total volume is the sum of their individual volumes.
- Mass (M): It’s the amount of matter in the system. Like volume, if you combine two systems, the total mass is the sum of the masses of each system.
- Internal Energy (U): This is the total energy contained within the system, including kinetic and potential energies of all particles. If two systems are combined, the total internal energy is the sum of their internal energies.
- Enthalpy (H): It’s the total heat content of a system. It’s defined as the internal energy plus the product of pressure and volume (H = U + PV). For two systems combined, the total enthalpy is the sum of their enthalpies.
- Entropy (S): This property measures the disorder or randomness in a system. When you combine two systems, the total entropy is the sum of their entropies.
These properties are important because they help describe the scale of the system and its capacity to contain matter and energy.
Solved Examples
Problem: A gas in a closed system expands from an initial volume of (2 m3) to a final volume of (5 m3) at constant pressure of (100 kPa). Calculate the work done by the gas.
Solution: The work done by the gas during expansion at constant pressure is given by:
\(\displaystyle W = P \Delta V \)
Given:
- Initial volume, (Vi = 2 m3)
- Final volume, (Vf = 5 m3)
- Pressure, (\(\displaystyle P = 100 \, \text{kPa} = 100 \times 10^3 \, \text{Pa}\))
Calculate the volume change:
\(\displaystyle \Delta V = V_f – V_i = 5 – 2 = 3 \, \text{m}^3 \)
Calculate the work done:
\(\displaystyle W = 100 \times 10^3 \, \text{Pa} \times 3 \, \text{m}^3 \)
\(\displaystyle W = 300 \times 10^3 \, \text{J} \)
\(\displaystyle W = 300 \, \text{kJ} \)
Therefore, the work done by the gas is (300 kJ).
Problem: Water flows into a heat exchanger at a rate of (2 kg/s). The inlet temperature of the water is (20∘C), and the outlet temperature is (60∘C). If the specific heat capacity of water is (\(\displaystyle 4.18 \, \text{kJ/kg} \cdot \text{K}\)), calculate the rate of heat transfer to the water.
Solution: The rate of heat transfer (\(\displaystyle\dot{Q}\)) in an open system is given by:
\(\displaystyle \dot{Q} = \dot{m} c \Delta T \)
Given:
- Mass flow rate, (\(\displaystyle\dot{m} = 2 \, \text{kg/s}\))
- Specific heat capacity, (\(\displaystyle c = 4.18 \, \text{kJ/kg} \cdot \text{K}\))
- Inlet temperature, (\(\displaystyle T_i = 20^\circ \text{C}\))
- Outlet temperature, (\(\displaystyle T_f = 60^\circ \text{C}\))
Calculate the temperature change:
\(\displaystyle\Delta T = T_f – T_i = 60 – 20 = 40 \, \text{K} \)
Calculate the rate of heat transfer:
\(\displaystyle \dot{Q} = 2 \, \text{kg/s} \times 4.18 \, \text{kJ/kg} \cdot \text{K} \times 40 \, \text{K} \)
\(\displaystyle\dot{Q} = 2 \times 4.18 \times 40 \)
\(\displaystyle\dot{Q} = 334.4 \, \text{kJ/s} \)
\(\displaystyle\dot{Q} = 334.4 \, \text{kW} \)
Therefore, the rate of heat transfer to the water is (334.4 kW).
Problem: A thermodynamic system undergoes a process where its internal energy increases by (500 J), and it does (200 J) of work on the surroundings. Calculate the heat added to the system during the process.
Solution: According to the first law of thermodynamics:
\(\displaystyle\Delta U = Q – W \)
Given:
- (\(\displaystyle\Delta U = 500 \, \text{J}\))
- (W = 200 J)
Rearrange to solve for (Q):
\(\displaystyle Q = \Delta U + W \)
\(\displaystyle Q = 500 + 200 \)
\(\displaystyle Q = 700 \, \text{J} \)
Therefore, the heat added to the system is (700 J).
FAQs
What distinguishes an open, closed, and isolated thermodynamic system?
An open system allows both energy and matter to be exchanged with its surroundings. A closed system allows only energy exchange but not matter exchange, while an isolated system allows neither energy nor matter exchange with its surroundings. These distinctions help in analyzing and understanding various thermodynamic processes.
How does the concept of entropy relate to thermodynamic systems?
Entropy is a measure of the disorder or randomness of a system. In thermodynamics, systems tend to move towards states of higher entropy over time. This means that processes within a system often lead to increased disorder or randomness, which is reflected in the increase of entropy. Understanding entropy helps in predicting the direction and feasibility of various thermodynamic processes.
Can you explain the significance of state variables in thermodynamic systems?
State variables, such as pressure, volume, temperature, and internal energy, describe the current state of a thermodynamic system. They are crucial because they uniquely define the system’s condition, regardless of the path it took to reach that state. By analyzing changes in state variables during different processes, we can understand and quantify the behavior of thermodynamic systems.
Why is it essential to consider the surroundings when analyzing thermodynamic systems?
Thermodynamic systems interact with their surroundings, exchanging energy and sometimes matter. Understanding these interactions is crucial for analyzing and predicting the behavior of the system. Additionally, considering the surroundings allows us to define boundary conditions and apply principles such as conservation of energy and mass, which are fundamental in thermodynamics.
How do phase transitions affect thermodynamic systems?
Phase transitions, such as melting, vaporization, and sublimation, involve changes in the state of matter within a thermodynamic system. These transitions typically involve the absorption or release of heat energy, leading to changes in the system’s internal energy and entropy. Understanding phase transitions is essential for various applications, from refrigeration systems to climate modeling.